lthough the word "language" is generally applied to human speech, it can also describe any form of expression in which information is transmitted and received. Scientists have learned that Nature abounds with communication emanating in many different languages -- from the vocal and non-vocal exchanges of mammals, to the chemical dialogues of plants and insects. Inanimate objects are not silent either. From the stars that light the heavens to the atoms that make up all of us and the world in which we live, science is a veritable gabfest of information ready to be shared with those who have learned how to listen.
Emerson saw the ideal use of language as a communication in which one's own nature is revealed, and from a scientific standpoint, few objects rival the atom when it comes to meeting Emerson's ideal. The chattiness of atoms with regard to their own nature and that of the molecules they form has found an eager audience in the scientific community. Any one of several different languages may be invoked to receive these communications, but perhaps none is potentially more revealing than "nuclear magnetic resonance" or NMR. To acquire this information, however, scientists must not only understand the language of NMR, they must also listen very carefully.
During the past two decades, one of the world's most avid NMR listeners has been Alexander Pines, a chemist with Berkeley Lab's Materials Sciences Division, and professor of chemistry on the Berkeley campus of the University of California. With consummate skill, Pines and his research group have coaxed atomic nuclei into an ongoing series of increasingly sensitive self-revelations. He has also been a major factor in expanding the scope of NMR listeners. For years, NMR was a language primarily useful only for the study of liquids. Beginning around 1967, when he was a graduate student working with John Waugh at MIT, and continuing since his arrival at Berkeley in 1972, Pines has helped extend the use of NMR to a broad range of solids and amorphous materials, organic as well as inorganic. Along the way he has worked closely with an ever-changing but consistently creative and dedicated group of graduate students and post-doctoral researchers (called the PINENUTS on their internet site), plus a long-standing core of collaborators, each an outstanding scientist in his own right.
To understand the value of NMR and how it works, remember that molecules are made up of atoms (e.g., a molecule of water is made up of one oxygen and two hydrogen atoms), and an atom consists of one or more negatively-charged electrons orbiting around a nucleus. An atomic nucleus is made up of one or more positively-charged protons and electrically-neutral neutrons. NMR is a phenomenon involving molecules whose atoms contain nuclei in which at least one proton or neutron is unpaired. The imbalance causes such nuclei to spin on an axis like miniature tops and gives rise to a magnetic moment, which means the nuclei act as if they were bar magnets with a north and south pole. (NMR was first observed nearly 50 years ago by physicists Felix Bloch of Stanford and Edward Purcell of Harvard, for which they won the 1952 Nobel prize in physics.)
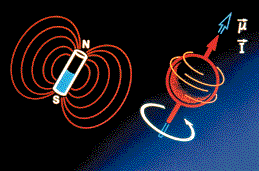 |
Under the effects of an external magnetic field, certain atomic nuclei will spin like a top about an axis. A spinning nucleus acts as if it has a north and south pole and attempts to align itself with the external magnetic field.
|
When exposed to a strong external magnetic field, these spinning "bar magnets" attempt to align their axes along the lines of magnetic force. The alignment is not exact, resulting in a wobbly rotation about the force lines that is unique for each type of nuclei. For example, hydrogen, which has a simple nucleus of one proton, rotates about the lines four times faster than carbon-13, whose nucleus is stuffed with six protons and seven neutrons. If, while exposed to the magnetic field, the nuclei are bombarded with radio waves, they will absorb and re-emit energy at a specific frequency according to their rate of rotation. This "resonating frequency" therefore becomes a signature signal by which the nuclei can be identified.
When nuclei absorb the energy of an incoming radio wave they are knocked out of alignment with the external magnetic field lines. As they lose this energy, the nuclei come back into alignment. The rate at which resonating nuclei realign themselves with magnetic field lines provides detailed information on their position and motion with regards to neighboring nuclei. Through NMR, scientists can study the structural, dynamic, and spatial relationships of atoms in a sample of molecules. No other means of communicating with atoms reveals so much without doing something that changes the nature of the sample.
"The spinning nuclei in a sample are like spies inside an unmapped and unexplored continent," Pines once said. "To learn about the interior of that continent, you don't have to destroy it, you don't even have to invade it, all you have to do is learn the spies' language and listen to them talk."
If NMR is viewed as a major language of atomic nuclei, then there are two dialects -- spectroscopy and imaging. In NMR spectroscopy, the frequency of the incoming radio wave is varied, and all of the different frequencies absorbed and emitted by the nuclei in a sample material are measured to obtain a resonance spectrum. This NMR spectrum reveals the molecular makeup of the material detailed down to the respective positions and motions of the constituent atoms. The second NMR dialect is used primarily in medicine, and also in biology and materials science, and goes by the name of Magnetic Resonance Imaging or MRI. In MRI, the frequency of the incoming radio wave is kept constant, but the strength of the external magnetic field is varied. The resulting signal corresponds to the total number of spinning nuclei present in any given part of a sample -- in other words, the atomic density of the sample at that point. This information can be translated by computer into a recognizable image, such as a picture showing the inside of a human brain or heart.
As a language, NMR is delivered at such a low volume it has always been difficult to detect and understand. This has been one of the major drawbacks for those who would use NMR. The problem is inherent and stems from the fact that when spinning atomic nuclei align themselves with the lines of a magnetic field, their axes point in either an "up" or "down" direction. The NMR signals from nuclei pointing in opposite directions cancel one another out, just as a positive electrical charge cancels out a negative charge. If a sample were to contain an equal number of nuclei with spins pointing up and down, no NMR/MRI signal would be obtained.
"The natural population difference between up and down nuclear spins is usually no more than one in 100,000 in NMR magnets at room temperature," explains Pines. "This low degree of spin polarization (meaning spin axes that point in a single direction) has been a persistent challenge for NMR."
Over the years, Pines and his many students and collaborators have been able to pump up the volume of NMR signals, particularly in solids, through a rich assortment of schemes which were given colorful names such as "multiple quantum NMR," "zero-field NMR," "double-rotation NMR" and "dynamic-angle spinning NMR." These and related techniques helped spread the NMR word into broad new areas of chemistry and biology where it had seldom if ever been used before. More recently, Pines and his collaborators have focused their efforts on the challenging problem of applying NMR to molecules in solution. They are overcoming this challenge through a technique called SPINOE (pronounced SPIN-OH-EE).
SPINOE stands for Spin-Polarization-Induced Nuclear Over-hauser Effect. In this technique, xenon gas, which has anesthetic properties, is specially treated so that it can amplify the volume of NMR signals from any atomic nuclei with which it makes contact. Using SPINOE NMR, Pines and his students, along with Gil Navon, a chemist with Tel-Aviv University, have been able to nearly double the strength of the NMR signals from hydrogen nuclei in solution.
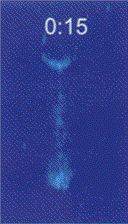 |
Hyperpolarized xenon gas introduced into a solution of benzene is shown over a period of 4 minutes. As the polarization from xenon nuclei is tranferred to the nuclei of the molecules in solution the NMR/MRI signals are amplified.
|
Xenon is an inert gas, meaning its molecules can make physical contact with other types of molecules without affecting them chemically. Xenon also readily dissolves in solutions, especially blood. This combination makes xenon safe and highly useful for medical studies. Xenon nuclei show a small degree of natural polarization in their spin, but zapping them with a beam of polarized laser light creates a "hyperpolarized" effect in which most of the spins point in the same direction.
The transfer of polarization from photons to the spins of atomic nuclei -- a process called "optical pumping" -- was first demonstrated by Alfred Kastler of the University of Paris who won the 1966 Nobel prize in physics for this research. A technique for optically pumping xenon (and helium as well) was subsequently developed by physicist Will Happer at Princeton University.
From Happer's work, it was shown that hyperpolarized xenon nuclei emit a strong NMR signal. Given this strong signal, it was the contention of Pines and Navon that if hyperpolarized xenon gas were to be bubbled into a solution, polarization from xenon nuclei would be transferred to the nuclei of molecules in the solution, amplifying their NMR/MRI signals.
To test this idea, the researchers introduced hyperpolarized xenon gas into a solution of benzene. Molecules of benzene are rich in hydrogen nuclei, which provide most of the NMR signals that yield MRI pictures. For the hyperpolarized xenon nuclei to influence the alignments of the hydrogen nuclei, they would have to override competing affects from the magnetic fields of other nuclei in the benzene molecules. This proved to be the case thanks to the accumulative effect of so many xenon nuclei pointing in the same direction. Pines likened the situation to a boxer whose punches are weak but who lands a lot of blows on the same spot.
"Every area in a molecule or sample accessed by the hyperpolarized xenon is going to light up to some extent with an enhanced NMR signal," Pines says.
Continue to part 2 of this story.